Neural Networks and Semantic Memory How Your Brain Creates Permanent Knowledge Structures
Neural Networks and Semantic Memory How Your Brain Creates Permanent Knowledge Structures - The Architecture of Neural Networks Inside Your Temporal Lobe
The intricate neural networks residing within the temporal lobe, particularly the medial temporal lobe (MTL), are fundamental to memory processing. The hippocampus acts as a central coordinator, integrating a vast network that underpins episodic memory throughout life. While the structural connections between neurons offer a basic understanding of communication pathways, a comprehensive grasp of memory requires delving deeper into functional connectivity. Simply mapping the physical connections isn't enough; the dynamic interplay between brain regions must be considered. For instance, semantic memory processing highlights the distributed nature of these networks, involving the temporal, parietal, and frontal lobes. This demonstrates the flexibility and adaptability of the brain's architecture. However, traditional notions of hierarchical information processing are being challenged by emerging hypotheses, like the "shallow brain hypothesis," which suggest that the brain might use different, perhaps simpler, computational strategies than previously imagined. This calls for continued exploration of the unique mechanisms at play within these neural pathways.
The temporal lobe, particularly the medial temporal lobe (MTL), stands out as a critical region for memory, especially the formation and recall of our knowledge base—semantic memories. There's a compelling overlap between how neuroscientists view the brain's structure and the way computer scientists build artificial neural networks. While we can map out the physical connections between neurons, simply understanding the network's wiring diagram doesn't fully illuminate how the brain actually functions. Functional connections—the patterns of neural activity—aren't just a byproduct of the physical layout.
The hippocampus, nestled within the MTL, is a central node in a complex network dedicated to episodic memory, suggesting its importance throughout our lives. Semantic memory, however, appears to be a more distributed phenomenon, relying on a broader network encompassing the temporal, parietal, and frontal lobes. This notion of a hierarchical network with various layers of abstraction is currently being debated. Some researchers believe that the brain, unlike many artificial networks, might operate on simpler, "shallower" principles.
Distinct pathways of interconnected neurons facilitate communication between specialized brain areas, creating a collaborative, dynamic system. Mapping the structure and function of specific MTL regions offers an avenue for deepening our understanding of how it works and may be crucial for diagnosing diseases associated with memory impairments. The hippocampus displays rhythmic brainwave patterns during different stages of memory processing—theta oscillations during encoding and synchronized bursts during rest, which may play a part in consolidating memories. This hints at intricate timing mechanisms, possibly a key component of memory formation, reminiscent of the importance of synchronization in artificial neural network designs.
There's a growing realization that studying the temporal lobe's internal architecture is vital for grasping its role in memory formation. This region's intricate design, including its layered structure with specialized neuron types, could be a significant factor in how we store and retrieve conceptual knowledge. Neuroimaging studies suggest that distinct parts of the temporal lobe are involved in processing different types of semantic information like words or images, showcasing a functional specialization, similar to how deep learning models use distinct layers for various tasks. Furthermore, the connection to the amygdala highlights the influence of emotions on memory, something that is still a developing area in artificial networks. Interestingly, the auditory processing in the temporal lobe, especially the superior temporal gyrus, underscores the sensory basis of memory, suggesting a strong tie between our senses and how we retain information—a vital lesson for anyone seeking to create more effective artificial neural networks.
Neural Networks and Semantic Memory How Your Brain Creates Permanent Knowledge Structures - Memory Proteins and Synaptic Plasticity in Long Term Learning
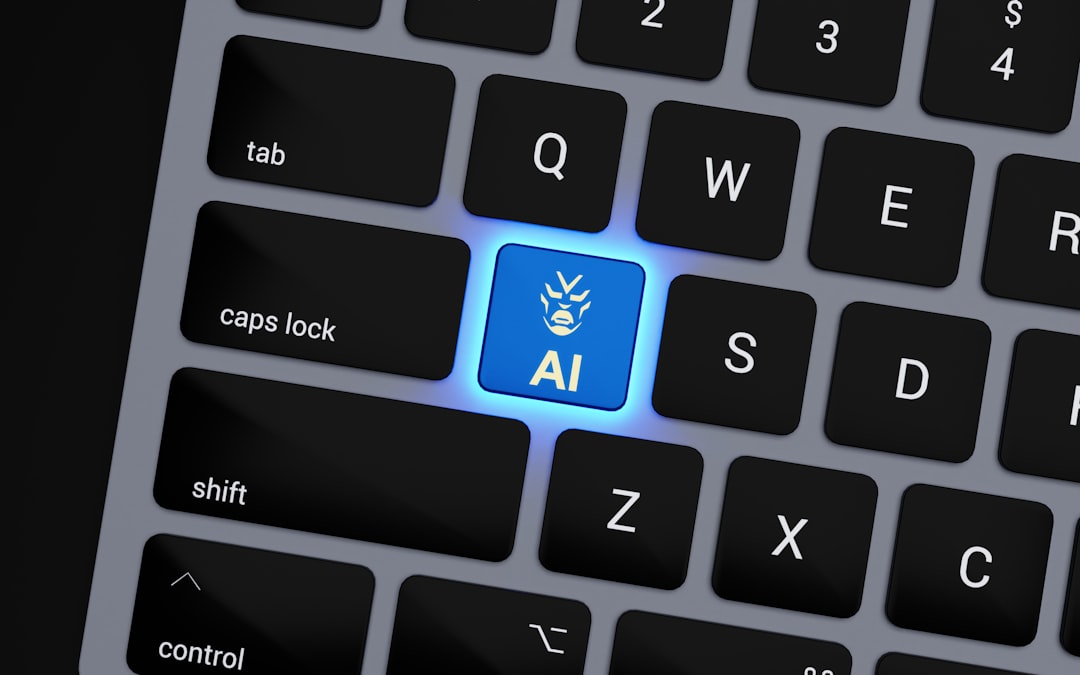
The ability to learn and retain knowledge over extended periods hinges on the interplay between memory proteins and synaptic plasticity. Synaptic plasticity, the brain's capacity to modify the strength of connections between neurons, serves as the cornerstone of long-term learning. This dynamic process allows for lasting alterations in synaptic strength, effectively shaping how our brains respond to new experiences. Long-term potentiation (LTP) emerges as a central player in this mechanism, especially within the hippocampus, a brain region pivotal for memory formation. LTP, a type of synaptic plasticity, boosts synaptic transmission, a key step in solidifying memories.
The brain's capacity for memory storage isn't uniform across regions. Diverse brain areas exhibit specialized forms of synaptic plasticity, highlighting the intricate network of processes involved in storing and retrieving long-term memories. Often, these lasting changes involve structural adjustments at the synapse—the junction between neurons—along with complex signaling pathways initiated by neurotransmitters. These pathways involve a cascade of molecular events, ensuring that the changes aren't fleeting. The intricate nature of these processes underscores how the brain constantly adapts to new information, allowing for continuous learning and knowledge integration throughout life. It also highlights the inherent complexity of the learning process and the remarkable plasticity of the human brain.
Synaptic plasticity, the ability of synapses to strengthen or weaken over time, is widely accepted as the foundation of long-term memory storage. This means that learning experiences can permanently alter the strength of connections between neurons. One key type of synaptic plasticity, called long-term potentiation (LTP), is particularly important in the hippocampus. LTP strengthens synaptic transmission, a process fundamental to memory consolidation—the transition from short-term to long-term memories.
However, it's not just the hippocampus that exhibits this flexibility. Different brain areas employ various forms of synaptic plasticity, suggesting a complex network where different circuits are dedicated to storing and retrieving long-term memories. Some types of synaptic plasticity involve physical changes in the synapse, coupled with intricate molecular processes. These processes often involve neurotransmitter systems, such as those using calcium ions (Ca2+), which help to cement memories.
Scientists frequently study LTP by simulating learning experiences using high-frequency electrical stimulation in neural pathways. This mimics how natural synaptic activity strengthens connections. The idea of "neurons that fire together wire together", rooted in Hebb's theory, emphasizes how synchronized neural activity can strengthen synapses during learning. This is a core principle for understanding how learning impacts our brain's physical structure.
Synaptic plasticity can be divided into presynaptic and postsynaptic forms. These involve different molecular processes, including mechanisms dependent or independent of the NMDA receptor. The hippocampus continues to be a critical area for memory research, acting as a focal point for understanding the link between synaptic plasticity and learning. This region is rich with molecular pathways that drive synaptic plasticity, including neurotransmitter systems and enzymes called kinases that are vital for consolidating memories.
It's fascinating that the ability to adapt learning through synaptic plasticity is seen across species. This adaptation underlies the development of intelligence and knowledge acquisition across a lifetime, both in humans and other animals. The ongoing research into how synapses change with experience highlights the constant adaptation of our brains, driven by our interactions with the world. The fine-tuning that occurs at the level of synapses is essential for how we learn and process information, a complex process that we are only just beginning to understand. It may also offer a foundation for improved AI designs that are inspired by biological processes. While research has yielded significant insights, we still have much to uncover concerning the intricate workings of these adaptive mechanisms within our brains.
Neural Networks and Semantic Memory How Your Brain Creates Permanent Knowledge Structures - Pattern Recognition Systems That Build Knowledge Frameworks
Pattern recognition systems are essential for constructing knowledge frameworks. They achieve this by employing sophisticated neural-like processes that discern and categorize information. These systems, analogous to the human semantic memory system, utilize predictive models to organize and retrieve interconnected knowledge structures. This highlights how concepts are linked and related. Contrary to older views of a fixed semantic memory, current understanding leans towards a more dynamic model where knowledge adapts based on the situation. This inherent flexibility complicates the precise identification of the neural components responsible for this adaptability, underscoring the complexity of cognitive processing. Ongoing research offers promising insights into the intricate mechanisms behind pattern recognition, potentially influencing the future development of more robust and adaptable artificial intelligence systems.
The way our brains structure knowledge might differ fundamentally from how we build artificial neural networks. Instead of the deep, hierarchical structures common in AI, the brain appears to leverage flatter, interconnected networks. This suggests a system optimized for both quick information processing and flexible adaptation, rather than rigid layered architectures.
Semantic memory isn't neatly packaged in one brain area, but rather distributed across multiple lobes. This dispersed architecture implies that knowledge is represented more like a complex web of associations instead of a straightforward, linear catalog. This presents a challenge to accurately modeling it.
Beyond static connections, brain regions communicate using intricate temporal patterns, the rhythm of neuron firing. This timing element profoundly affects how information gets processed, akin to the temporal coding techniques found in some advanced artificial networks that handle sequential data.
The brain's memory retrieval isn't static—it's deeply context-dependent. Certain brain networks get activated based on environmental cues, mirroring the context-aware capabilities of certain machine learning algorithms. However, replicating the sophistication of human context-awareness is proving challenging.
Memory is surprisingly dynamic: every time we recall a memory, it's subject to modification before being stored again—a process known as reconsolidation. This constant updating gives us adaptable knowledge frameworks but also presents challenges for both the accuracy of human memory and the reliability of AI systems that try to model such frameworks. This area needs further research.
Different types of neurons in the MTL handle specific aspects of memory processing. This specialization within the network suggests that the brain enhances its capabilities by incorporating specialized elements—similar to how complex AI systems use different algorithms for different tasks.
The brain expertly weaves together different sensory inputs during memory formation, like blending visual and auditory data. This cross-modal integration highlights the brain's sophisticated ability to connect information types, an area that many artificial systems struggle to replicate effectively, despite our advances in sensor technology.
The impact of various neurotransmitters on synaptic strength is nuanced, unlike the more static learning rates in some artificial networks. Serotonin, dopamine, and other neurochemicals contribute to complex regulatory mechanisms in memory formation, presenting a frontier in neuro-inspired computing.
The brain employs fascinating mechanisms, like synaptic scaling, to maintain stability during learning. This prevents information overload and facilitates continuous knowledge acquisition. AI developers are working towards implementing countermeasures in deep learning to avoid saturation as new information is added. It's not simple.
The collaboration between the hippocampus and the amygdala showcases how our emotions can deeply influence memory encoding and retrieval. This aspect, strongly connected to how our experiences are associated with various feelings, remains mostly unexplored in artificial systems that often focus on primarily logical data processing. This is a large area for future research and improvement in AI systems.
Neural Networks and Semantic Memory How Your Brain Creates Permanent Knowledge Structures - Distributed Networks vs Single Location Memory Storage
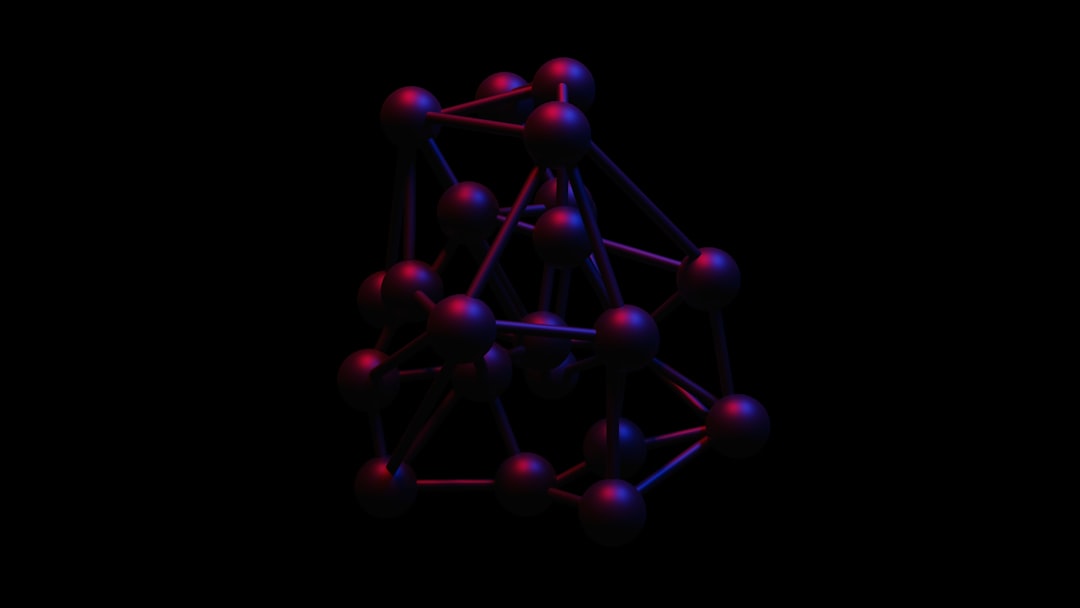
The question of how our brains store and retrieve semantic memories—the foundation of our knowledge base—has led to a critical discussion about distributed networks versus single-location storage. The idea that memory resides in one specific area is being challenged by growing evidence that semantic memory is, in fact, spread across various interconnected brain regions. This distributed nature, encompassing the temporal, parietal, and frontal lobes, suggests a collaborative system rather than a hierarchical one with a central command center. This distributed architecture allows the brain to adjust how it recalls information based on the situation and what task is at hand. The flexibility offered by a dispersed memory system is a powerful advantage for adaptation and learning. On the other hand, the concept of a single storage location for memory becomes less tenable as our understanding of memory's context-dependent and dynamic nature grows. Recognizing this distinction between a centralized versus a networked approach is becoming increasingly important for fields like cognitive neuroscience and AI, as we strive to create computational models that more accurately reflect the brain's intricate memory processes.
In contrast to the idea of a singular memory storage location, the brain's semantic memory system is a marvel of distributed networks. This means knowledge isn't confined to a single area but is spread across various brain regions. This distributed architecture has several advantages. For one, it enhances resilience. If one part of the network is compromised, the remaining regions can still access and process information, making the system more robust to errors or damage. It also seems to improve the efficiency of retrieving memories, potentially by allowing for parallel access to multiple pieces of knowledge at once.
Furthermore, this distributed model paves the way for more effective problem solving. By leveraging the parallel processing capacity of multiple brain areas, we can potentially tackle complex problems from different angles concurrently. This inherent parallelism promotes creative solutions and a flexibility that traditional, singular storage systems might struggle with.
This decentralized structure also gives the brain an incredible degree of adaptability. Our ability to continuously learn and adjust based on new experiences hinges on this distributed design. It's what allows us to navigate ever-changing environments and evolve our understanding of the world. Static memory systems, on the other hand, struggle to incorporate new knowledge seamlessly.
Beyond mere storage, the distributed nature of semantic memory also incorporates sophisticated timing mechanisms. Rather than just storing data points, the network employs rhythmic patterns and timing of neuron firing to encode information. This adds a temporal dimension to memory, mirroring how some advanced computing systems handle real-time data. This dynamic aspect suggests that time and context are critical components of how the brain processes information.
This network of interconnections within the brain isn't a simple hierarchical structure but more resembles a web. This complex web provides a vast capacity for forging associations between seemingly disparate concepts, creating rich semantic frameworks that go far beyond simple linear classifications.
Another intriguing aspect of distributed networks is memory reconsolidation. Every time we access a memory, it undergoes a reconsolidation process, a phase where it's essentially updated and re-stored. This dynamic process makes memories malleable and subject to change with each retrieval. It's a hallmark of human cognition that's not readily found in traditional memory models.
Furthermore, the distributed model incorporates specialization within the network. Distinct populations of neurons within the distributed network are responsible for processing particular types of information. For instance, different areas of the brain handle auditory and visual memories, despite their interconnectedness. This specialization potentially leads to more powerful and nuanced representations of knowledge compared to a simplistic, singular memory repository.
The influence of neurochemicals on memory within these distributed networks is also striking. While artificial systems often have static learning rates, the complex interplay of neurotransmitters like serotonin and dopamine dynamically modulates memory strength and retrieval. This modulation enhances the brain's ability to prioritize memories based on emotional relevance, a crucial factor in human experience.
Furthermore, distributed networks handle sensory integration remarkably well. They seamlessly blend visual, auditory, and other sensory data, creating richer and more holistic memories. This stands in contrast to single-location models, which might struggle to combine information from multiple sensory sources cohesively.
Finally, understanding the distributed nature of memory systems is crucial for research into disorders affecting memory. By taking into account this complex architecture, we can gain a deeper understanding of cognitive impairments. This perspective is vital, as simplistic models of memory can potentially misrepresent or oversimplify the intricacies of memory dysfunction.
In essence, the distributed nature of semantic memory provides a remarkable framework for cognition, encompassing adaptability, resilience, complex information processing, and dynamic memory reconsolidation. It's a paradigm that challenges conventional notions of memory and presents a powerful lens for understanding the human mind and its extraordinary ability to create and maintain knowledge.
Neural Networks and Semantic Memory How Your Brain Creates Permanent Knowledge Structures - Memory Consolidation During Sleep and Knowledge Formation
Sleep plays a vital role in solidifying our knowledge by consolidating memories, essentially transforming fragile, short-term memories into more stable, long-term ones. This process, referred to as memory consolidation, involves a complex interplay of activities within the brain during sleep. One key aspect is the phenomenon of synaptic downscaling, where the strength of connections between neurons is adjusted to optimize the storage of newly acquired information. Simultaneously, the brain engages in neural replay, effectively rehearsing recently experienced patterns of activity to strengthen the relevant memory circuits. These processes are further facilitated by the optimal neurophysiological environment created during sleep, promoting a heightened state of brain plasticity—the brain's ability to change and adapt.
Intriguingly, recent evidence also links dreaming to this memory consolidation process. While the exact function of dreaming is still under investigation, it seems to serve as a period where recent memory traces are refined and reorganized for long-term storage within our knowledge networks. This consolidation process might be a critical step in the formation of the intricate knowledge structures that underpin our understanding of the world.
Furthermore, the field of targeted memory reactivation (TMR) has emerged as a powerful tool to study memory processing during sleep. By manipulating memory cues during sleep, researchers have been able to explore the underlying mechanisms involved in sleep-based memory consolidation. This rapidly developing field offers a unique window into how our brain constructs its knowledge, potentially impacting how we understand cognitive health and optimize learning. This active research points towards the profound role sleep plays in our ability to acquire and retain knowledge, making it essential for both cognitive function and optimal learning.
Sleep's influence on memory formation is becoming increasingly clear, with evidence from both human and animal studies consistently pointing towards its crucial role in long-term memory. One of sleep's key functions during brain development is to support experience-driven changes in the brain, or neural plasticity, which are critical for building and refining memories and shaping future sleep patterns. It's important to note that "sleep-dependent memory consolidation" is a broad term encompassing various memory types and processes.
A deeper look at the mechanisms suggests that the consolidation of memories primarily reliant on the hippocampus seems connected to the activation of a signaling pathway involving a specific protein, Erk12 MAPK. This pathway plays a vital role in the genetic processes, transcription and translation, which are necessary for establishing long-term memories. This leads us to the understanding of memory consolidation during sleep as an active process of restructuring existing neural connections. This process involves reducing overall synaptic activity, which happens across the brain and is helped by the brain's repetition of hippocampal patterns during sleep.
The consolidation of memories also seems to have a strong influence on the content of our dreams. Recent memory traces are thought to be actively stabilized and reorganized in a way that prepares them for long-term storage. This link is suggestive of an intricate interplay between memory and the sleeping mind. It also seems that the environmental conditions during sleep, the neurophysiological aspects, play a substantial role in how effectively memories are consolidated and how brain networks are strengthened.
Memory creation relies on this malleability of the brain's structure and function, that is brain plasticity. It is the lasting changes in the structure and function of neurons that occur due to experiences. It seems that a specific experimental technique, targeted memory reactivation (TMR), offers a promising path forward in understanding the process of memory consolidation. It is based on manipulating memory processing during sleep, and it has given us insights that we may not have gotten otherwise. A quick review of published research reveals a rapidly growing area of study, with over 70 publications focusing on TMR in just the last decade, underscoring the importance of understanding how sleep affects memory.
While there have been significant strides in understanding sleep's role in memory, we still face challenges in precisely defining how it works, including clarifying the exact roles of different brain regions and neurochemicals in the process. The complex interplay between various neural processes, genetic mechanisms, and environmental conditions makes understanding sleep-dependent memory consolidation a multifaceted research endeavor. It is clear however that understanding this interaction is fundamental to a deeper understanding of how the brain shapes knowledge. It seems highly plausible that further investigation into these mechanisms may uncover further strategies for enhancing cognitive abilities and potentially for improving artificial systems.
Neural Networks and Semantic Memory How Your Brain Creates Permanent Knowledge Structures - Why Emotional Connections Create Stronger Memory Structures
The strength of our memories is profoundly impacted by the emotional weight we associate with them. This heightened memory retention stems from a collaborative effort between the hippocampus, a key region for memory formation, and the amygdala, the brain's emotional hub. When we recall emotionally-charged events, neurons within the hippocampus fire in a synchronized pattern, effectively strengthening those memories. Conversely, neutral experiences don't trigger the same level of coordinated neural activity, making them less likely to be firmly etched in memory.
Furthermore, the amygdala's influence is crucial in shaping how we encode and retrieve emotional memories. It orchestrates various processes that contribute to the strength and durability of emotional memories, highlighting the link between the intensity of our emotional responses and the enduring nature of the memories they create.
The field of emotional memory is expanding to include the social context of our experiences. How we interact with others, the relationships we build, and the emotions associated with these connections can all influence our memory formation. This suggests a complex web of factors, both emotional and social, that determine how we remember things.
The intricate way in which the brain handles emotional memories represents a fertile ground for continued research within cognitive neuroscience. Further understanding of these processes has the potential to inform the development of more advanced artificial intelligence systems that can more accurately capture the complexity of human memory and emotional processing.
The interplay of emotions and memory formation is a fascinating area of research, particularly in the context of understanding how the brain constructs knowledge. We know that emotional events, compared to neutral ones, tend to be etched more vividly into our memories. This enhanced memory encoding seems tied to the amygdala, a brain region that plays a key role in processing emotions. It appears the amygdala helps to consolidate memories that are emotionally salient, making them stick around longer.
Neurochemicals, such as dopamine and norepinephrine, appear to play a crucial role in how emotions influence memory. When we experience emotionally arousing situations, levels of these chemicals increase, potentially strengthening the synaptic connections involved in forming those memories. It's plausible that these stronger connections contribute to the greater durability of emotional memories.
Interestingly, even when we recall an emotional memory, our brain doesn't just replay it. There's a process called reconsolidation where the memory is, in essence, updated based on our current emotional state. This means that past memories can be modified by our present emotions, which might introduce potential biases into our knowledge structures.
The context surrounding an emotional memory also influences how we recall it. It's similar to how some machine learning algorithms use context to improve predictions. This suggests a degree of cognitive flexibility, where the brain can adapt how it accesses memories based on the surrounding environment or current task.
Furthermore, sleep seems to have a specific role in strengthening emotional memories, in addition to its general role in consolidating memory. During sleep, our brain reactivates patterns of activity linked to recent emotional experiences, effectively strengthening the underlying neural circuits.
The collaboration between the hippocampus and the amygdala is essential in this process. The hippocampus helps with forming the memory of the context and details of the event, whereas the amygdala provides the emotional "tag" to that memory. This dual-processing system seems necessary to create robust and nuanced memory networks.
Interestingly, research indicates that we can retrieve emotional memories more quickly than neutral ones. This rapid retrieval might enhance decision-making, particularly in high-stakes situations, by providing swift access to relevant past experiences.
Emotional experiences also seem to encourage deeper processing of information. This deeper level of encoding, bolstered by emotional involvement, could impact the pathways used for retention and retrieval of the memory.
Human social interaction also seems to play a role in memory, specifically emotional memory. When our emotions are related to social situations, areas of the brain associated with empathy and memory are stimulated. This suggests that the richer the tapestry of social interactions, the stronger the memory structures can potentially become.
There's a delicate balance at play. While emotional engagement can enhance memory, situations involving high cognitive load during emotional experiences can potentially hinder the memory consolidation process. This suggests a limit to the brain's capacity for integrating both emotion and information during intense situations.
This field continues to evolve as we uncover more about the intricate relationship between emotion and memory. It's a critical avenue for research, as understanding this connection is fundamental to gaining a more comprehensive understanding of human cognition and its interplay with the brain's intricate architecture. It may also offer opportunities for improving cognitive function or potentially informing designs for more human-like AI.
More Posts from psychprofile.io: